Take a deep breath. What if I told you the thing you had to thank for being able to do that was a microscopic organelle called a chloroplast, would you believe me? Well believe it!
Chloroplasts are vital for the existence of life on Earth, they are small structures responsible for an important process called photosynthesis. Photosynthesis is the process of converting sunlight to breathable oxygen but the process, as you will see in this post, is much more complex than it seems.
Chloroplasts are organelles restricted to plants, algae and some protists. Like mitochondria, chloroplasts also have a double membrane, ribosomes and their own DNA that has the function of synthesizing the necessary proteins to perform photosynthesis.
In this post we will look at chloroplasts in detail and some of the processes and chemical reactions that make the photosynthetic process possible!
Structure and Function
Chloroplasts vary in size from 5 to 10 micrometers long and they have a double membrane called the chloroplast envelope and a third internal membrane known as the thylakoid membrane. The thylakoid membrane forms an extensive network of flattened discs called thylakoids, and these are organized in stacks called grana.
The chloroplast’s membranes divide the organelle into three sections. The first is the intermembrane space between the inner and outer membrane, the second is the stroma (a fluid rich in soluble proteins and ribosomes located between the inner membrane and the thylakoid membrane) and the third is the thylakoid lumen (the inside of the thylakoids) (figure 1) (Cooper, 2000).
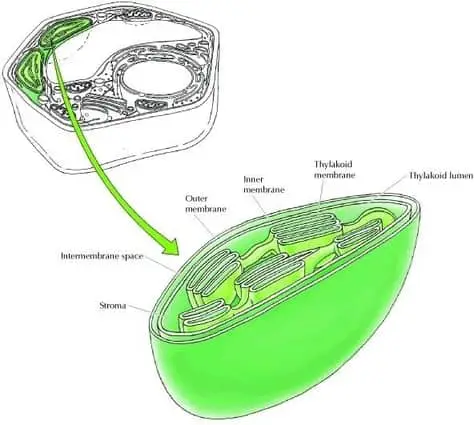
Chloroplasts have evolved into their function of absorbing sunlight and carrying out photosynthesis reactions. Starting with the outermost part, the inner membrane has the function to be highly selective in what molecules allows to enter and leave the organelle, meanwhile, the stroma (where the light-independent reactions take place) houses the chloroplast DNA and ribosomes, which are capable of manufacturing several proteins needed in photosynthesis, as well as all the enzymes needed to carry out the light-independent reactions.
Chloroplasts can move! Chloroplasts have been observed moving by way of cytoplasmic streaming. This is process where the chloroplasts are transported in the cytoplasm presumably to a position for optimal light absorption. The speed at which this transport happens can vary based on a number of different factors like, temperature, pH levels, and light exposure.
Last but not least the grana (where light-dependent reactions take place) provide a large surface area where photosynthetic pigments (chlorophylls and carotenoids), electron carriers and enzymes (like ATP synthase that helps to manufacture ATP) are attached. These photosynthetic pigments are held by a network of proteins forming special light collecting units called photosystems, which are essential to the photosynthesis process (Toole & Toole 2004).
History
In 1665 Robert Hooke developed a compound microscope with magnifying lenses and was capable of observing the arrangement of cell walls from plant tissue. Shortly after, Nehemiah Grew was the first to observe chloroplasts in his research about the microanatomy of plants where he reported a green matter in plant leaves.
But it wasn’t until 1837 when the botanist Hugo von Mohl provided the first description of chloroplasts (named chlorophyllkörnen in German) as discrete bodies within the plant cell. Later, the botanist Andreas Schimper named these bodies plastids but at the end of the century, the term chloroplast had become generally accepted.
However, the structure of chloroplasts remained murky until the discovery of the electron microscope in the mid-20th century which allowed researchers to describe individual membranes, chloroplasts ribosomes, fibrous material identified as DNA and other discrete structures (Hoober 1990).
Similarity with Mitochondria
A major point in the development of life as we know it was the oxygenation of the atmosphere due to the evolution of prokaryotes capable of photosynthesizing molecular oxygen approximately 2 billion years ago.
The most accepted theory suggests that an endosymbiotic event between an ancestral eukaryotic cell and an aerobic prokaryote occurred. This gradually led to the development of a mitochondrion allowing eukaryotic cells to be aerobic. Then, eukaryotic cells containing mitochondria went through a second endosymbiotic event with cyanobacterium that evolved into chloroplasts.
As we can see, both mitochondria and chloroplast have a similar origin, characteristics and functions, such as the presence of outer and inner membranes, and their own ribosomes and DNA that allow them to transform energy into a form that the cell can use. However, they differ in many ways too.
Chloroplasts are only found in plants, they contain different structures (like thylakoids and stroma), and pump protons across the membrane using the sun’s energy and produce oxygen (Rogers 2010, Johnson 2016). While there are some functional similarities and a possible evolutionary convergence, it seems that chloroplast and mitochondria should be thought of as distant cousins rather than twins.
Photosynthesis
We definitively cannot talk about chloroplasts without explaining photosynthesis. Photosynthesis is the process that sustains life on Earth, providing the oxygen that we need to breathe and the food we need to live. In fact, cyanobacteria, diatoms, dinoflagellates and other phytoplankton produce nearly 50% of all oxygen generated by primary producers on Earth.
Photosynthesis occurs because of two reactions that take place in different parts of the chloroplasts called light and dark reactions. The light reactions, also known as light-dependent reactions, take place on the thylakoid membranes where light is used to break down water into oxygen, protons, and electrons.
The dark reactions, also known as light-independent reactions, occur in the stroma where the protons and electrons transferred through the thylakoid membrane create ATP (Adenosine triphosphate) and NADPH (Nicotinamide adenine dinucleotide phosphate), which are then used to reduce CO2 to a carbohydrate (figure 2) (Johnson 2016, Suthers et al. 2019). The chemical equations for the described reactions involved in photosynthesis are the listed below:
Light reactions:
2H2O + light → O2 + 4H+ + 4e−
Dark reactions:
CO2 + 4H+ + 4e− → CH2O + H2O
Overall: H2O + light + CO2 → CH2O + O2
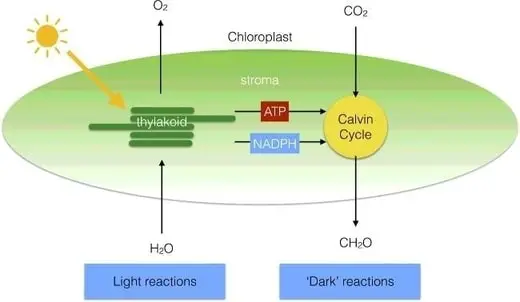
Light-dependent reactions start with the absorption of light by photosynthetic pigments. The two most important pigments are chlorophylls and carotenoids. Chlorophylls are a group of green pigments of which chlorophyll a and chlorophyll b are the most important ones while carotenoids are yellow, orange and red pigments which are known as accessory pigments because their contribution to light reactions is indirect.
This happens because carotenoids absorb certain light wavelengths more efficiently than chlorophylls, so they pass the captured energy to chlorophyll a for it to be used in the light-dependent reactions. When photosynthetic pigments absorb photons, a higher energy state is created that converts the energy of sunlight to chemical energy.
This happens because of groups of photosynthetic pigments (located in the thylakoid membrane) function as antennae that absorb light and transfer the energy of their excited electrons to a reaction center (a complex of proteins and photosynthetic pigments), where the signal is converted into a different form (figure 3) (Toole & Toole 2004, Blankenship 2014).
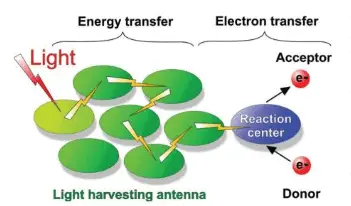
There are two reaction center complexes, the photosystems I and II (also known as P700 and P680, respectively, due to the maximal absorption of light reach by the chlorophylls associated to each photosystem). The process of converting the sunlight energy to chemical energy begins in the photosystem II where the energy acquired from the photon absorption is used to split the water, oxidizing it to molecular oxygen that is then released as a waste product.
The electrons extracted from water are carried by a plastoquinone to the cytochrome bf complex responsible for the transfer of electrons to a membrane protein (called plastocyanin) causing 4 protons to be pumped into the thylakoid lumen. The electron transport chain continues with the electrons transferred from the plastocyanin to the photosystem I, where light absorption generates high energy electrons that are transferred to ferredoxin (a small protein outside thylakoid membrane) that can form a complex with the enzyme NADP reductase to reduce NADP+ to NADPH (figure 4).
As we can see, the electron transfer through the photosystem II establishes a proton gradient that drives the synthesis of ATP by the ATP synthase enzyme and the electron transfer through the photosystem I and drive the NADPH synthesis. Both ATP and NADPH are then used in the light-independent reactions (Toole & Toole 2004, MacAdam 2009, Blankenship 2014, Cooper 2019).
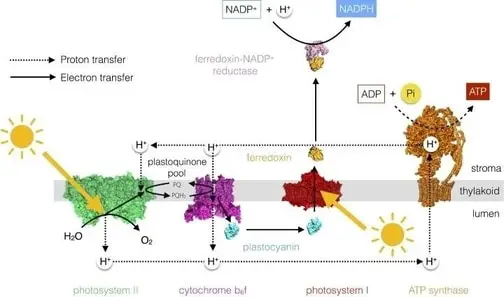
The light-independent reactions drive the production of glucose from CO2 and H2O in the chloroplast stroma. These reactions are light-independent because they don’t require light directly and can occur whether or not it is available.
However, these reactions require the product from the light-dependent reactions, ATP and NADPH. The cycle of reactions where a molecule of CO2 leads to the formation of carbohydrates is known as the Calvin cycle (named after his discovered Melvin Calvin).
Briefly, the carbon dioxide diffuses from the atmosphere through the stroma where it combines with ribulose phosphate using the enzyme ribulose bisphosphate carboxylase (also known as rubisco) to form an unstable compound that breaks down into two molecules of 3-phosphoglycerate, which are then converted into glyceraldehyde-3-phosphate using ATP and NADPH.
From 12 molecules of this metabolite, 2 molecules are then used to produce glucose while the other 10 are used to regenerate ribulose phosphate by the use of ATP (figure 5). Essentially, the Calvin cycle consumes 18 molecules of ATP, 12 molecules of NADPH and 24 electrons for each molecule of glucose produced (Toole & Toole 2004, Cooper 2019).
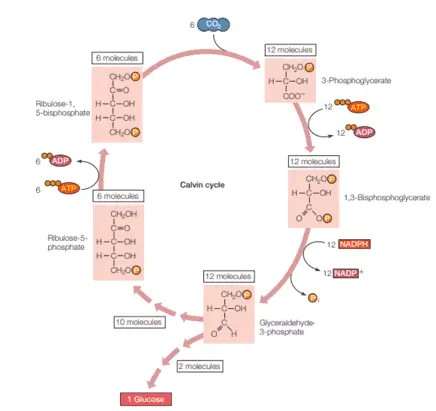
Chloroplasts and Greenhouse Gasses
Human activities and other natural factors have increased the CO2 concentration in the atmosphere since the Industrial Revolution began, which has altered the global carbon cycle. The use of fossil fuels results in greenhouse gas emissions, specifically CO2, methane, nitrous oxide and fluorocarbons. The increase in the concentration of greenhouse gases is at least partially responsible for the rise in Earth’s temperature.
Scientists are studying the use of photosynthetic organisms as one possible solution among many to help mitigate CO2 emissions. Photosynthesis is a natural process that drives the fixation of atmospheric CO2, and rubisco is a key enzyme in this process responsible for fixing 250 billion tons of CO2 per year. Fixation of atmospheric CO2 means transforming inorganic CO2 to organic CO2 so it can be used by organisms.
Despite the ability of plants, algae, and cyanobacteria to absorb CO2 from the atmosphere, several studies have shown that algae have higher growth potential and efficiency in their photosynthetic apparatus compared to other organisms. For example, its efficiency in reducing CO2 is 10-50% higher than terrestrial plants.
In this way, the role of algae in the reduction of greenhouse gas emissions have been studied with promising results. For instance, species from the Euglena genus have been related with an increase in the absorption of CO2 emissions in the atmosphere through carbon dioxide fixation and with the decrease of methane emissions from livestock after using them as part of animal feed.
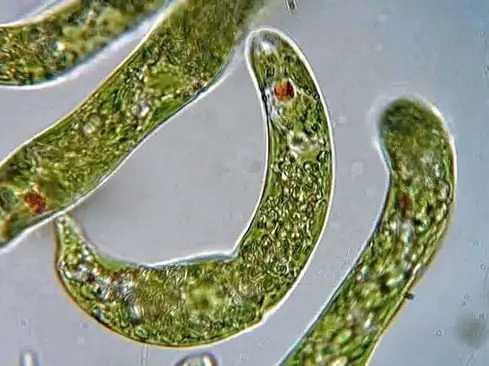
In addition, algae are capable of producing biofuels by the accumulation of lipids and other compounds that store energy. Several algae species produce up to 60% of neutral lipids per gram of dry weight, which makes them one of the most efficient biofuel production systems. In summary, the development of new technologies that improve the use of algae can potentially be used to mitigate the impact of climate change (Sayre 2010, Aemiro et al. 2016, Bracher et al. 2017, Asadian et al. 2018).
Chloroplasts and Other Organisms
Even though photosynthesis only occurs in plants, algae, and cyanobacteria, several of these photosynthetic organisms have some interesting relationships with other organisms.
Kleptoplasty
Kleptoplasty is a symbiotic phenomenon that occurs when algae’s chloroplasts are ingested by other organisms but are not digested, remaining structurally intact and photosynthetically competent for long periods of times, in some cases, even for the entire lifespan of the organism.
The word kleptoplasty is derived from the Greek [kleptes] which means ‘thief’. The name was appropriately chosen because the chloroplasts are sequestered by a new host. This phenomenon has been reported in distantly related lineages such as dinoflagellates, ciliates, foraminifers and metazoans (Serôdio et al. 2014). Once inside the new host, the chloroplasts are called kleptoplasts.
Depending on the host species the kleptoplasts remain functional for different periods of time. An example can be found in the research of Gast and collaborators, where they studied how much time dinoflagellates could survive without additional chloroplast intake. Their results showed that some dinoflagellates can retain kleptoplasts for 1 month, while others for up to 8 months.
Another example extensively studied is the symbiosis with sea slugs. These organisms feed on macroalgae and sequester their chloroplasts in tubule cells of their digestive system (figure 6); Laetz & Wägele reported that two species, Elysa timida and Elysa cornigera survived 42 and 29 days respectively, after being deprived of food.
These researchers also found that the survival of the same species varies according to temperature revealing the complex processes behind kleptoplasty in these animals (Gast et al. 2007, Serôdio et al. 2014, Laetz & Wägele 2018).
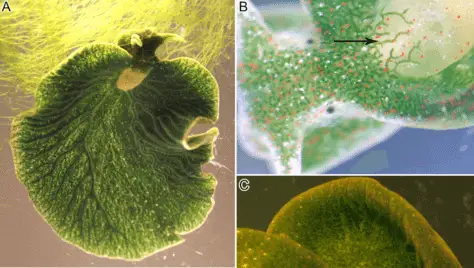
Symbiosis
The symbiosis between animals and photosynthetic symbionts appears to be limited to aquatic invertebrates, the reported hosts include sponges, corals, anemones, turbellarians, marine gastropods and bivalves and ascidians.
However, one of the most interesting examples symbiosis occurs between corals and dinoflagellate microalgae known as zooxanthellae. This association has been successful due to the benefits that zooxanthellae provide to corals, such as higher calcification rates, higher productivity and survival, in fact, zooxanthellae provide more than 90% of coral’s nutritional requirements.
This obligated symbiosis has been maintained for more than 200 million years, which is the time reefs have been dominated by zooxanthellate corals (Fabricius et al. 2004, Ulstrup et al. 2006, Venn et al. 2008, Allemand & Furla 2018). The coral-zooxanthellae relationship is highly sensitive to stress, especially increases in temperature that causes the disruption of the symbiosis and becomes white by the loss of zooxanthellae.
This coral bleaching usually happens when seawater temperature increases between 1-2°C above to the max temperature reached in summer if the thermal stress lasts for several months or escalate the corals die. The high temperatures cause damages in photosystems II of the zooxanthellae resulting in the reduction of photosynthesis, disruption of coral growth and loss of energy reserves.
Interestingly, when corals with and without bleaching belonging to the same species were seen in the same reef, studies were conducted to know why; it turns out that several clades of zooxanthellae are associated with corals and the thermal tolerance is not the same for all. (Fabricius et al. 2004, Ulstrup et al. 2006, Lewis et al. 2019).
Takeaways
To sum up, chloroplasts are incredible organelles responsible for producing oxygen and carbohydrates from sunlight, CO2 and water, through the process of photosynthesis. The fact that CO2 fixation occurs in chloroplasts allows all photosynthetic organisms to be potentially able to help reduce the CO2 emissions in the atmosphere.
However, as the characteristics of each group are different, the use of microalgae has come up as a promising and efficient possibility to mitigate the impact of climate change, especially with the reduction of greenhouse gases emissions.
The ability to produce energy with sunlight presents an amazing advantage and this is probably the reason why so many successful examples of symbiosis with photosynthetic organisms are reported. Certainly, chloroplasts are of tremendous importance going from small processes that only affect within a cell to large scale processes that affect the development and continuation of life on the planet.
References
- Aemiro, A., Watanabe, S., Suzuki, K., Hanada, M., Umetsu, K., & Nishida, T. (2016). Effects of Euglena (Euglena gracilis) supplemented to diet (forage: concentrate ratios of 60: 40) on the basic ruminal fermentation and methane emissions in in vitro condition. Animal Feed Science and Technology, 212, 129-135.
- Allemand, D., & Furla, P. (2018). How does an animal behave like a plant? Physiological and molecular adaptations of zooxanthellae and their hosts to symbiosis. Comptes rendus biologies, 341(5), 276-280.
- Asadian, M., Fakheri, B. A., Mahdinezhad, N., Gharanjik, S., Beardal, J., & Talebi, A. F. (2018). Algal communities: An answer to global climate change. CLEAN–Soil, Air, Water, 46(10), 1800032.
- Blankenship, R. E. (2014). Molecular mechanisms of photosynthesis. John Wiley & Sons. 312 pp.
- Bracher, A., Whitney, S. M., Hartl, F. U., & Hayer-Hartl, M. (2017). Biogenesis and metabolic maintenance of Rubisco. Annual review of plant biology, 68, 29-60.
- Cooper, G. M. (2000). The Cell: A Molecular Approach. 2nd edition. Sunderland (MA): Sinauer Associates. Chloroplasts and Other Plastids.
- Cooper, G. M. (2019). The Cell: A Molecular Approach. 8th edition. Oxford University Press. 813 pp.
- Fabricius, K. E., Mieog, J. C., Colin, P. L., Idip, D., & H. Van Oppen, M. J. (2004). Identity and diversity of coral endosymbionts (zooxanthellae) from three Palauan reefs with contrasting bleaching, temperature and shading histories. Molecular ecology, 13(8), 2445-2458.
- Gast, R. J., Moran, D. M., Dennett, M. R., & Caron, D. A. (2007). Kleptoplasty in an Antarctic dinoflagellate: caught in evolutionary transition? Environmental Microbiology, 9(1), 39-45.
- Hoober, J. K. (1990). Chloroplasts. Springer Science & Business Media. 280 pp.
- Johnson, M. P. (2016). Photosynthesis. Essays in biochemistry, 60(3), 255-273.
- Laetz, E. M. J., & Wägele, H. (2018). How does temperature affect functional kleptoplasty? Comparing populations of the solar-powered sister-species Elysia timida Risso, 1818 and Elysia cornigera Nuttall, 1989 (Gastropoda: Sacoglossa). Frontiers in zoology, 15(1), 1-13.
- Lewis, C., Neely, K., & Rodriguez-Lanetty, M. (2019). Recurring episodes of thermal stress shift the balance from a dominant host-specialist to a background host-generalist zooxanthella in the threatened pillar coral, Dendrogyra cylindrus. Frontiers in Marine Science, 6, 5.
- MacAdam, J. W. (2009). Structure and function of plants. John Wiley & Sons. 304 pp.
- Pelletreau KN, Weber APM, Weber KL, Rumpho ME (2014) Lipid Accumulation during the Establishment of Kleptoplasty in Elysia chlorotica. PLoS ONE 9(5): e97477.
- Rogers, K. (Ed.). (2010). The cell. Britannica Educational Publishing. 240 pp.
- Sahoo, D., Elangbam, G., & Devi, S. S. (2012). Using algae for carbon dioxide capture and biofuel production to combat climate change. Phykos, 42(1), 32-38.
- Sayre, R. (2010). Microalgae: the potential for carbon capture. Bioscience, 60(9), 722-727.
- Serôdio, J., Cruz, S., Cartaxana, P., & Calado, R. (2014). Photophysiology of kleptoplasts: photosynthetic use of light by chloroplasts living in animal cells. Philosophical Transactions of the Royal Society B: Biological Sciences, 369(1640), 20130242.
- Suthers, I., Rissik, D., & Richardson, A. (Eds.). (2019). Plankton: A guide to their ecology and monitoring for water quality. CSIRO publishing. 248 pp.
- Toole, G., & Toole, S. (2004). Essential A2 biology for OCR. Nelson Thornes, Cheltenham, UK. 176 pp.
- Ulstrup, K. E., Ralph, P. J., Larkum, A. W. D., & Kühl, M. (2006). Intra-colonial variability in light acclimation of zooxanthellae in coral tissues of Pocillopora damicornis. Marine Biology, 149(6), 1325-1335.
- Venn, A. A., Loram, J. E., & Douglas, A. E. (2008). Photosynthetic symbioses in animals. Journal of experimental botany, 59(5), 1069-1080.